Index
Group Alexander Kaier
Food security, consumer preferences, and the imminent threats of the global climate change represent strong forces for breeders to find fast and efficient ways to develop new crop varieties accordingly. As a result, a precise and deep knowledge of abiotic stress resistance and the underlying genetic factors in plants is of utter most importance to find new targets for crop improvement.
The aim of our research is the analysis of different major and minor crop plants to reveal genomic and transcriptomic variations leading to improved crop performance under various stress conditions. To identify these factors we use state-of-the-art statistical and bioinformatic tools from the field of quantitative and population genetics. In addition, we take advantage of the increasingly powerful machine learning technologies. Together, these tools are used to analyze and integrate diverse data sets such as genomics, transcriptomics, metabolomics, and phenomics. This helps us to disentangle gene regulatory networks and the genetic basis of complex traits and to find new targets for molecular breeding.
Currently, our research focuses on the multi-omics analysis of potato (Solanum tuberosum). It is one of the most important food crops globally and ranks third only behind the cereals rice and wheat. Due it’s steady increase in overall production it is considered an important staple food source. The modern cultivars originated from the Andes in South America between Bolivia and Peru. Domestication of wild potato dates back nearly 8,000 years. Cultivated potatoes were introduced into Europe in the 1570s and were distributed globally in the late 17th century. Today, potatoes are grown world-wide from latitudes of 65 ⁰N to 50 ⁰S and from altitudes of up to 4,000 m. This demonstrates the immense adaptability of potato to many different environmental conditions. Even though potato is well adapted to a variety of environmental conditions, it shows a high sensitivity to abiotic stress conditions such as heat and drought. Especially high temperature (temperatures above 20 ⁰C) impacts tuber production of potato negatively.
Due to the vastly changing climate, increasing global population, and the necessity to produce food even under suboptimal conditions, we are working with national and international partners to improve potato in one main projects. For details on each project please feel free to contact the PhD student working on the project or our group leader (Alexander Kaier).
- Epigenetics of heat tolerance in potato (Darren Yeo)
During the last decade, it is becoming increasingly clear that agronomic traits of crops such as tuber size and yield are inextricably linked with epigenetic regulation because of environmental stresses. Epigenetic gene regulation refers to the regulation of gene expression by modifying the genome without altering the sequences in the genome. One such epigenetic modification is the methylation and demethylation of cytosines. Methylation patterns may differ among different genotypes, individual plants or even among the tissues and are often associated with environmental stresses including heat stress. This phenomenon could potentially be true for potatoes as well when grown under heat stress. Thus, we aim to profile the methylation patterns among genotypes that show contrasting behavior regarding their heat tolerance. Differences in the methyliation patterns may help illuminate the mechanisms governing heat adaptation in this important crop.
“CASS Transformation and Research Lab“ – Group Christian Lamm
As part of the CASS-project, the CASS Transformation and Research Lab works on the development of in vitro methods enabling genetic transformation of agriculturally relevant Cassava genotypes, and routinely provides our project partners with genetically modified Cassava plants.
Plant biotechnology is indispensable for modern plant research and breeding. For instance, it can be used to elucidate the molecular functions of enzymes and proteins, improve existing metabolic pathways, or introduce entirely new ones. In many cases, even modern genome editing techniques such as the CRISPR/Cas system rely on methods of classical genetic engineering.
However, the lengthy procedures require precise adaptation to the plant of interest. In fact, even within a species, only few cultivars may be transformed efficiently. A major disadvantage, especially when it comes to genetic modification of crop plants: Accessible cultivars do not necessarily meet agricultural needs, for example due to lower yields or higher susceptibility to pathogens or abiotic stress. The emergence of genome editing techniques has particularly led to increased research efforts aiming at transformation of agriculturally useful varieties of various crops.
In times of global climate change and increasing population, plant biotechnology alongside modern breeding techniques could make a crucial contribution to urgently needed global food security.
Within the CASS project, we are addressing this issue with the tropical crop Cassava, which is mainly grown by smallholder farmers in Sub-Saharan Africa for its starchy storage roots. However, drastically lower yields are achieved here compared to other regions, mainly due to the lack of availability of fertilizers, biocides, and modern agricultural equipment. Therefore, our stated goal is a biotech-driven yield stabilization in low input environments.
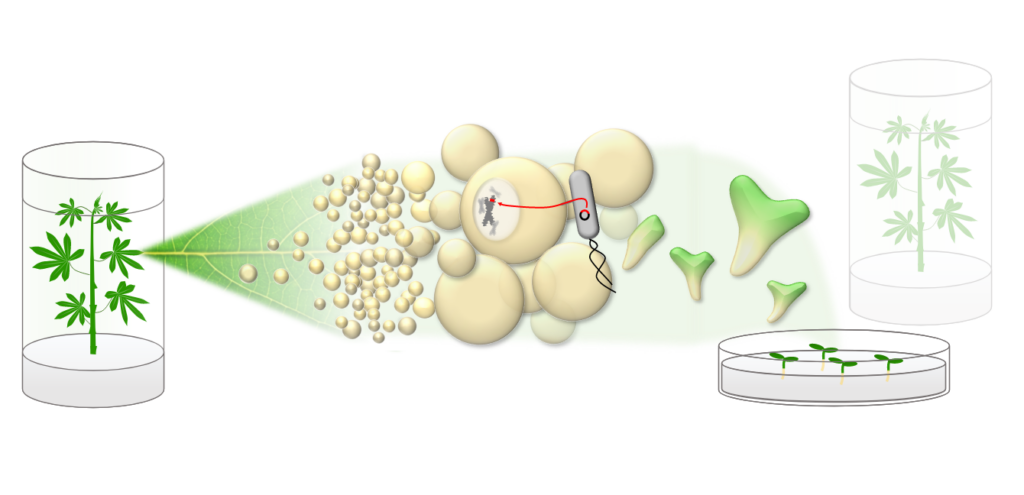
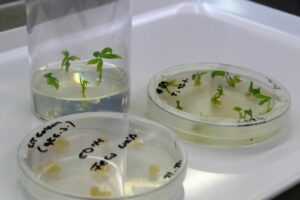
The CASS Transformation and Research Lab is aiming to improve the genetic transformation of Cassava in order to be able to modify farmer-preferred varieties. We investigate chemical media additives that alter the developmental program of plant cells (e.g. by targeting the epigenetic regulation) and thus enable transformation and/or regeneration.
Additionally, we work on genetic elements influencing the transformation and regeneration processes, as the ectopic expression of certain developmental regulators can be used to genetically modify cultivars that were previously not amenable to transformation – a principle that other research groups have already applied successfully in crops such as maize.
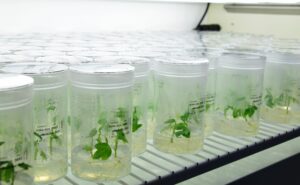
In addition to these research questions, we perform routine transformations of the Cassava model genotype TMS60444 for our project partners, covering the entire workflow (Fig.1): Cassava plants are grown under sterile conditions and treated with phytohormones to produce dedifferentiated callus tissue.
A suitable transformation plasmid is generated through molecular cloning and introduced into a lab strain of the soil bacterium Agrobacterium tumefaciens. The bacterium is naturally capable of introducing genetic material into plant cells, and through cultivation with the callus tissue, targeted transfer of the desired DNA can occur.
By altering the media composition, fully formed plants with the desired genetic material integrated into their own genome can be regenerated (Fig.2). After molecular confirmation of genomic integration, the plants are vegetatively propagated and can be analyzed further (Fig.3,4).
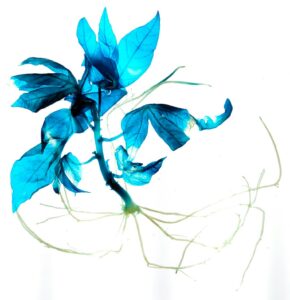
“Cassava Source-Sink“ – Group Wolfgang Zierer
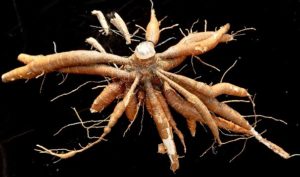
We strive to improve cassava storage root yield. Cassava is a tropical crop that is consumed by over 800 million people worldwide and is of special importance for the food security of smallholder farmers in Sub-Saharan Africa.
The plant grows up to 3-4 meters in height and generates several starchy storage roots under good conditions. These storage roots (Fig. 1) and their starch are the basis for a multitude of foods.
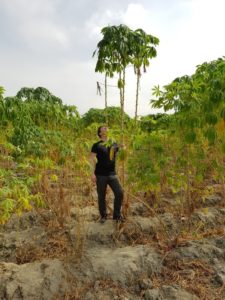
Alongside many other options, the storage roots can be consumed as vegetables in cooked or fried state or their starch can be processed into a variety of products, like the African Gari, a popular kind of puree.
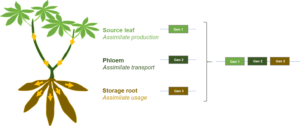
IUnfortunately, cassava yield is still comparably low in contrast to the heavily yield-optimized crops like wheat or maize. A sustainable cassava yield improvement would therefore have a large impact on the food security of millions of people. Therefore, we dedicate our work towards cassava yield improvement.
This goal is unfortunately not within easy reach and a biotechnological improvement of cassava yield is dependent on a very detailed understanding of the plant. Therefore, our work also contains a lot of basic science to unravel the physiological and biochemical processes of this exiting plant species.
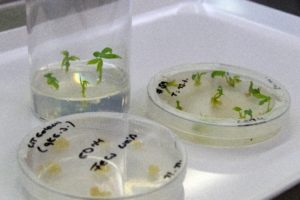
We mainly research the regulation of carbohydrate metabolism in auto- and heterotrophic tissues, assimilate transport, as well as developmental processes. Within these processes, we are trying to identify genes with positive impact on storage root yield and to combine them eventually for even larger impact (Fig. 3).
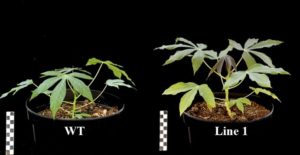
We test our target genes via transgenic cassava plants, which we generate ourselves or with the help of our collaboration partner at the ETH Zurich. Cassava transformation comprises refined tissue culture protocols, which can regenerate entire plants from dedifferentiated plant cells (Fig. 4).
As a first step, we test our modified cassava plants with various techniques in the greenhouse. Plants that can outperform their unmodified genetic background are of course of special interest for us (Fig. 5).
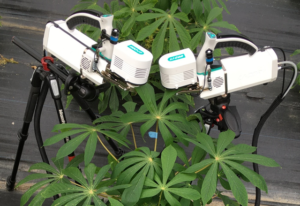
In addition to the determination of agronomic parameters and different physiological experiments like gas-exchange measurements (Fig. 6), we routinely employ most modern techniques of molecular plant research, like transcriptome- and proteome studies (Fig. 7).
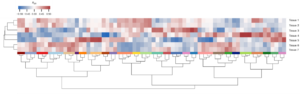
Several metabolite measurements are part of our routine application as well. Enzymatic or chromatographic sugar measurements for instance, grant us insight into primary plant metabolism (Fig. 8).
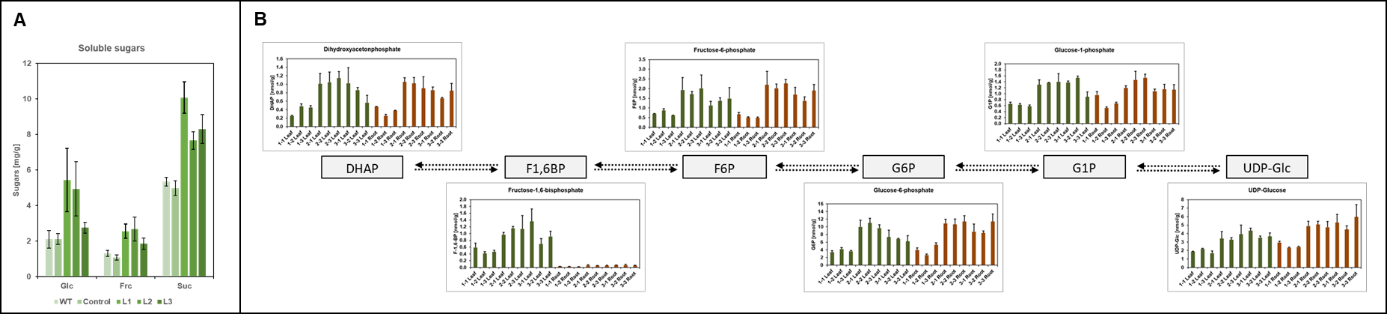
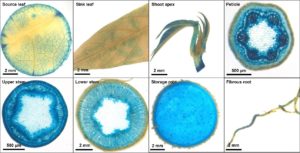
In addition to the identification and analysis of various genes, we also work on the improvement of tools for the generation of better transgenic cassava plant. Examples are better transformation protocols or the identification of tissue specific promoter sequences (Fig. 9)
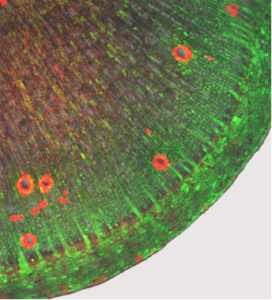
Furthermore, we conduct different studies on cassava wild type plants and various breeding material to understand underlying physiological processes, like the storage root formation or the assimilate transport.
Recently, we could clarify the symplasmic connections in cassava stems and storage roots by introducing and following fluorescent substances into the plant (Fig. 10).
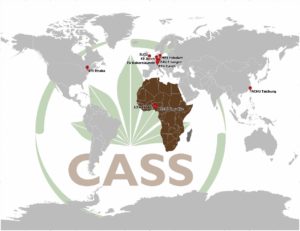
Our research group is part of the Cassava Source-Sink (www.cass-research.org) project (CASS) and has excess to a large network of plant science techniques and expertise through our various partners (Fig. 11).
We have close collaborations with:
- International Institute of Tropical Agriculture (IITA), Bioscience, Ibadan, Nigeria
- National Root Crops Research Institute (NRCRI), Umudike, Nigeria
- Chung-Hsing University (NCHU), Advanced Biotechnology Center, Taichung, Taiwan
- Sainsbury Laboratory Cambridge University (SLCU), Cambridge, United Kingdom
- Boyce Thompson Institute, Plant Research (BTI), Ithaca NY, USA
- Eidgenössische Technische Hochschule (ETH), Chair of Biochemistry, Zurich, Switzerland.
- Technische Universität Kaiserslautern, Chair of Plant Physiology, Germany
Fig. 12: Different phases of field-testing at NCHU Taichung, Taiwan. - Forschungszentrum Jülich, Institut für Bio- und Geowissenschaften, Germany
Together with our partners, we established an entire plant-testing pipeline, starting with the generation of transgenic cassava plants, followed by laboratory and greenhouse pre-testing, and ultimately field-testing (Fig. 12).
Overview of a young cassava field
Overview of an advanced cassava field
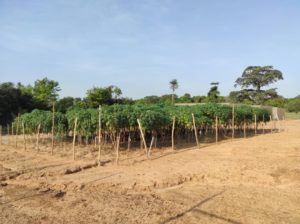
Our field trials are executed together with NCHU Taichung, Taiwan and the IITA Ibadan, Nigeria (Fig. 13).
We test transgenic cassava plants with alterations in source-sink metabolism at both location. IITA Ibadan also does conventional cassava breeding and we work together on different projects characterizing these genotypes.
Our research group and the entire Cassava Source-Sink project hope to contribute towards food security of African smallholder farmers. If you are interested in cassava or would like to support us in any way, feel free to contact wolfgang.zierer@fau.de.
Climate Adaptation of Potato – Group Sophia Sonnewald
My research group is mainly interested in the regulation of source – sink interaction during plant development and by adverse environmental conditions, such as heat & drought. We are using mainly potato plants as a model system. Potato plants are among world’s most important crops. Their tubers are an excellent staple food as they are rich in starch and contain minerals, vitamins and essential amino acids. However, potato plants are sensitive to heat and drought and global climate changes are expected to largely affect yield stability and tuber quality.
Our current research focuses on two main topics:
- Understanding responses of potato plants to heat and drought to improve them for the challenges of climate change
- Molecular analysis of potato tuber development
Improving potato plants for the future challenges of climate change
Elevated temperatures affect many physiological and developmental processes in potato plants. Among those are a reduced photosynthetic assimilate production and a strong negative effect on tuber development, starch accumulation and quality.
An important regulator of these processes is the Flowering Locus T homolog SP6A, which is a key tuberization gene. Its gene expression is reduced by heat coinciding with decreased tuber growth. In recent work, we uncovered a small RNA (termed SES) that is strongly induced by elevated temperatures and targets SP6A for post-transcriptional degradation (Lehretz et al. 2019; see Fig. 1).
- In ongoing work (DFG HotNet; https://gepris.dfg.de/gepris/projekt/432435747) we will further uncover the regulatory network acting under heat stress in potato plants by combining physiological and biochemical with molecular and genetic approaches. We exploit the genetic variability ( 2) to elucidate target genes as we think that there is a great potential to increase yield stability using the available genetic resources. Potato is a complex, highly heterozygous tetraploid crop that renders simple genetic approaches more difficult. Together with the bioinformatics group of Stephan Reinert we perform a detailed phenotyping of various tetraploid cultivars and using a GWAS approach together with transcript profiling experiments identify candidate genes.
- Within the Horizon 2020 EU funded project Adapt, we aim at a better understanding of responses of potato plants to combined stresses in particular to heat, drought and waterlogging. Apapt is a research consortium of 17 partners from leading academic research institutions, potato breeders, a non-profit EU association, a government agency and a plant phenotyping and imaging technology developer. The objectives are to identify new breeding targets and potato varieties that allow adaptation to changing environmental growth conditions in the future. Amongst others, we will further analyse the role of SP6A and the small regulatory RNA SES. For more information and updates visit the official project’s website (adapt.univie.ac.at) and Twitter account (@eu_Adapt).
- Changing environmental conditions also lead to reduced tuber starch content and may cause second tuber growth (Fig. 2b, c). Both traits result in loss of tuber quality and hamper their usage. Therefore, my group seeks after elucidating the underlying molecular mechanisms.
Molecular analysis of potato tuber development
- Potato tuber development strongly depends on metabolic and developmental signals from the leaves. Thus, the photoperiodic pathway with Constans / SP6A as key regulators has a strong control over tuber formation and growth. Recent work uncovered that SP6A interacts with sucrose efflux transporter(s) such as Sweet11b and may thereby promote assimilate translocation towards developing tubers. Even though our knowledge increased recently, there are still many open questions about the mode of action and the endogenous and environmental regulators.
Bioanalytics – Group Jörg Hofmann
The Bioanalytics group established a Metabolomics technology platform and a Proteomics platform enabling the qualitative and quantitative analysis of biomolecules. This includes targeted and untargeted metabolite profiling. The Metabolomics branch consists of the following equipment:
Metabolomics platform
LC/MS-MS System
- ABI/MDS-Sciex QTRAP-3200 (Mass Spectrometer)
- Dionex ICS-3000 Ion Exchange HPLC (metabolite analysis)
- Dionex ULTIMATE-3000 HPLC (metabolite analysis)
Other instruments
- Dionex SUMMIT P680 HPLC (metabolite analysis)
- Shimadzu GCMS-QP2010S mit GC-2010 (Fatty acid analysis)
- Äkta Purifier HPLC (Protein purification)
- Pharmacia P-800 FPLC (low pressure LC)
- Packard Flow Scintillation Analyser (Radioactive molecules)
- BIO-TEK ELISA Multiwell microplate photometer (Vis fluorescence)
- Goebel UVIKON-XL Spectrophotometer (UV/Vis)
Access
Prior to use the analysis platform the requirements of our regulations (PDF, german) apply and a sample submission form which is available on request must be filled in and sent via e-mail to: joerg.hofmann (at) fau.de
The core of our LC-MS/MS system represents the QTrap-3200 which is a versatile hybrid mass spectrometer. It combines the features of three cascaded mass filters (Triple-Quadrupole) needed for precise quantification with the concept of a linear iontrap enabling to derive information about quality (structure determination). This combination cares for an enhanced sensitivity. The Triple-Quadrupole together with other technical features (eg. N2-curtain) allows the analysis of relatively crude samples. For sensitive molecules we can adjust the harshness of ionisation by switching between different ion-sources ( Gas assisted Electro Spray Ionisation (ESI), Atmospheric pressure chemical ionisation (APCI) ). The ion source receives the stream of analytes after separation by the ICS-3000– ion chromatography- or the Ultimate-3000 HPLC system.
The inert ICS-3000 IC is exceptionally suited for ion exchange chromatography e.g. of carbohydrates or phosphorylated intermediates. It generates a “reagent-free” eluent required for mass spectrometry and has additional detectors (conductivity- and 3D amperometry detector) e.g. for the screening of sugars. In combination with the QTrap mass spectrometer we can use this device for targeted metabolite profiling with higher dynamic range and a broad spectrum of compounds.
Combining the Ultimate 3000 HPLC with an UV/Vis Photodiode Array Detector (PDA) results in a sophisticated 3D-visualization of fluorescence signals allowing easy identification and sensitve quantification of e.g. pigments, such as carotenoids or chlorophyll.
We have an additional HPLC system (Dionex SUMMIT) with a fluorescence detector suitable for the sensitive measurement of compounds like tocopherol and (derivatized) amino acids after Reversed Phase (RP) Chromatography.
We are also currently improving methods for GC/MS-based determination of fatty acids.
Our newly installed laboratory is further equipped with various supporting devices. Two types of absorption photometers are used to measure e.g. sugar concentration: 96-well ELISA Plate Readers with washer and fluorescence-detector facilitate quick simultaneous analyses of multiple samples. UV/Vis Spectrophotometers allow more sensitive absorption measurements.
In combination with our Flow Scintillation Analyser after chromatographic separation, we can detect radioactively labeled compounds. Furthermore we have installed a cold lab with an Äkta purifier and a low pressure FPLC sytem for the separation of proteins.
This equipment allows the parallel investigation of metabolites in complex mixtures and supports projects aimed at investigating plant-microbe interactions and primary plant metabolism ( AG S.Sonnewald, AG U.Sonnewald ). Furthermore, plant-cell-to-cell connections (plasmodesmata) are analysed at the biochemical and molecular level.
Proteomics platform
As part of Zentralprojekt Z1 in the Sonderforschungsbereich SFB796 a Proteomics lab was established in 2014. Identification and analysis of proteins and protein complexes with their post-translational modifications can be performed with mass spectrometry instruments.
This platform consists of Ultimate 3000 nano-HPLC and Orbitrap Fusion Tribrid systems designed for proteomic bioanalytics research. Optimized procedures of sample preparation and analysis enable us to work effectively with purified fractions, intact proteins, SDS-PAGE bands/spots, immunoprecipitated proteins (on/off-beads) for the identification of
- Interacting partners
- PTMs
- Differences at protein level (e.g. wild type vs. mutation, transfection, differential expression, stress influence)
- Proteome/Secretome studies
Special projects like Biomarker discovery, quantification (labelled/label-free) and phosphoproteome analysis are under development.
Operation and management of the platform is conducted by Jörg Hofmann
For further details please contact: joerg.hofmann (at) fau.de , uwe.sonnewald (at) fau.de
Sample submission form to apply for a measurement:
Download this PDF into a file, fill it in, print it into a PDF file again and send it by e-mail.
Plant-Biochemistry and Biotechnology – Group Uwe Sonnewald
The working group is concerned with application-oriented basic research in the areas of plant growth and development as well as aspects of synthetic biology. Plant growth is regulated by internal and external factors, and anticipated climate changes pose a major challenge for agriculture. In this context, we investigate the molecular basis of plant adaptation to periods of heat and drought in order to make them fit for climate change. In order to increase the productivity of crops such as potatoes or cassava, we are pursuing a biotechnological approach to improve assimilate production, distribution and utilization. In the field of synthetic biology, we are trying to reprogram plant metabolism through targeted protein-protein interactions so that it is better adapted to future climate conditions. At the heart of this work are intermolecular, break-resistant and specific protein linkages ( doi: 10.1186/s13007-020-00663-9 ; doi: 10.1371/journal.pone.0179740 ).
Adaptation to climate change:
According to independent climate models, a redistribution of annual precipitation and an increase in mean temperature in Germany can be expected within the next few decades. However, little research has been carried out to determine which adaptation mechanisms can protect crops particularly effectively against combined heat and drought stress. Even a moderate increase in atmospheric temperature can have drastic effects on the yield potential of potato plants. In this context, the impact on tuber formation plays a key role (doi.org/10.1146/annurev-arplant-080720-084456 ).
In order to better understand the molecular background of drought- and heat-induced yield losses, different potato genotypes are grown under controlled greenhouse and field conditions in international and national research projects and their yield development under stress is comparatively analyzed. Through the combination of genotyping by sequencing, molecular, biochemical, physiological and agronomic phenotyping, yield-relevant genome regions are predicted and validated. This identifies relevant processes, genes and alleles that can be used for breeding and biotechnological purposes. In addition, specific studies are being carried out on known processes. Current results indicate that elevated temperatures suppress the expression of a tuber-inducing FT signal, which greatly reduces tuber formation ( doi: 10.1111/pce.13366 ). The FT protein known as SP6A (Self-Pruning 6A) belongs to the Phosphatidyl Ethanolamine Binding Proteins (PEBP) which, among other factors, support the assimilate supply of tubers by inhibiting the activity of SWEET proteins ( doi: 10.1016/j.cub.2019.02.018 ). By specifically influencing the expression of SP6A, we are looking for ways to produce potato genotypes that can withstand the expected climate change. Both traditional breeding and biotechnological (genome editing, transgenic plants) methods are being used to achieve this goal. Promising results of transgenic SP6A overproducing potato plants, which are characterized by increased tuber formation, show that this could indeed be successful (doi.org/10.1016/j.jplph.2021.153530). Similar to the analyses on heat tolerance, we are trying to improve the potato’s adaptation to drought stress. To this end, we are pursuing approaches to optimize water loss through transpiration or water uptake by improving root growth. In this context, the combined expression of a guard cell-specific hexokinase and SP6A has produced the first potato plants that can withstand combined drought and heat under greenhouse conditions (doi.org/10.3389/fpls.2020.614534). These promising results provide the basis for further research that will hopefully lead to climate-adapted potato varieties.
Improving source-sink relations in crop plants:
The yield of crop plants is largely determined by the interplay between leaves and storage organs. The leaves convert atmospheric carbon dioxide into organic carbon compounds (a process called photosynthesis), whereby oxygen is produced as a “waste product”. Since mature leaves produce more carbohydrates during the day than they consume at night, they can make the surplus available to other organs. The leaves are referred to as source organs. The excess organic compounds are transported from the leaves via the plant’s vascular system to consuming or storing tissues (sink organs). Once in heterotrophic tissues, the compounds are either used for growth (e.g. roots) or storage (in seeds, tubers, etc.). The more assimilates are used to build up storage substances, the greater the yield. Therefore, the interests of plant breeding are focused on storing as many assimilates as possible in storage organs. Based on an increasing understanding of the processes involved in both leaf metabolism and storage metabolism, a number of biotechnological approaches have been developed that promise to increase crop yields (summarized in doi.org/10.1016/j.molp.2022.11.015). The ratio between total biomass and harvestable biomass is referred to as the harvest index. As part of two international research projects ( www.photoboost.org ; cass-research.org ), we are specifically trying to change the interaction between the leaf and storage root of cassava plants and between the leaf and storage tuber of potato plants so that higher yields can be realized. The focus here is on the simultaneous improvement of both the leaf metabolism (source), the transport (phloem) and the metabolism of the storage roots/tubers (sink). The approaches of the cassava project are summarized in the publication by Sonnewald et al., 2020 ( doi: 10.1111/tpj.14865 ). Higher-level approaches are described in Fernie et al, 2020 ( doi: 10.1038/s41477-020-0590-x ).